Lithium-ion batteries have been attracting attention since they were put on the market in 1991 because of their high energy density and long life. It has become an indispensable part of the energy economy in the 21st century. However, there are still some issues that need to be addressed urgently in the application of lithium-ion batteries in the field of large-scale batteries such as automobiles and energy storage, such as safety issues. The organic electrolyte of lithium-ion batteries is volatile, flammable and explosive, and is the primary element that causes safety problems in lithium-ion batteries. The all-solid-state lithium-ion battery has dealt with this problem from the root, and it also has the advantages of large capacity and light weight. It is urgent to study the all-solid-state lithium-ion battery that can be industrialized.
1. Overview of all solid-state lithium-ion batteries
All-solid-state lithium batteries are relative to liquid lithium batteries. They refer to energy storage devices that do not contain liquid in the structure and all materials exist in solid state. Specifically, it is composed of positive electrode material + negative electrode material and electrolyte, while the liquid lithium battery is composed of positive electrode material + negative electrode material + electrolyte and separator.
Research progress of all solid-state lithium-ion batteries
As the central component of the all-solid-state lithium-ion battery-the lithium-ion solid electrolyte material is the central material to complete its high function, and it is also one of the bottlenecks affecting its practicality. The development history of solid electrolytes has surpassed one hundred years. There are hundreds of solid electrolyte materials being studied. As long as the conductivity of solid electrolytes at room temperature or not too high temperature is greater than 10-3S/cm, it is possible to apply Electrochemical power supply system, and the conductivity value of most materials is several orders of magnitude lower than this value, which makes there are few solid electrolyte materials with practical application value.
2. Research progress on solid electrolytes
As a vital component of the battery, the function of the electrolyte largely determines the power density, cycle stability, safety function, high and low temperature function and service life of the battery. The indicators for judging electrolytes generally include:
(1) Ionic conductivity: Ionic conductivity will affect the body resistance of the assembled battery. For solid electrolytes, the ionic conductivity is generally required to reach 10-4S/cm or more.
(2) Relocation number: refers to the share of lithium ion contribution in the current passing through the electrolyte. Ideally, the relocation number is 1. If the relocation number is too low, anions will be enriched on the surface of the electrode, which will increase the polarization of the battery and increase the resistance.
(3) Electrochemical window: The electrolyte needs high electrochemical stability within the working voltage range of the battery, otherwise it will be decomposed during the working process. Generally, the electrochemical window is required to be higher than 4.3V.
The solid electrolytes currently being studied mainly include oxide solid electrolytes, sulfide solid electrolytes, polymer solid electrolytes, and composite solid electrolytes. These solid electrolytes and their research progress will be introduced in detail below.
Research progress of all solid-state lithium-ion batteries
2.1 Oxide solid electrolyte
According to the material structure, the solid oxide electrolyte is divided into crystalline electrolyte and glassy (amorphous) electrolyte. Crystalline electrolytes include Garnet type solid electrolytes, perovskite type Li3xLa2/3-xTiO3 solid electrolytes, NASICON type Li1+xAlxTi2-x(PO4)3 and Li1+xAlxGe2-x(PO4)3 solid electrolytes. The glassy electrolyte includes an inverse perovskite-type Li3-2xMxHalO solid-state electrolyte and a LiPON thin-film solid-state electrolyte.
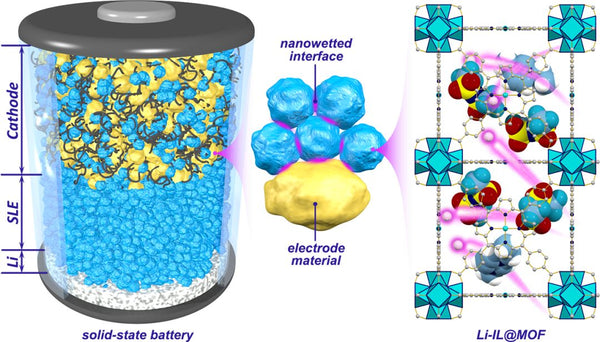
2.1.1 Garnet type solid electrolyte
The traditional Garnet-type electrolyte is Li7La3Zr2O12 (LLZO). The cubic-phase Garnet-type electrolyte has a higher room temperature ionic conductivity (10-3S/cm) and is more stable than other types of electrolytes when it touches metal lithium. It is currently considered to be one of the more promising electrolytes.
At present, Garnet-type electrolytes face two major problems:
1. The higher lithium content makes the surface of the electrolyte easily interact with water and CO2 in the air to generate lithium hydroxide and lithium carbonate, which then leads to a larger interface impedance, which makes the battery function worse.
2. Garnet-type electrolyte has poor wettability with metallic lithium. Lithium ions accumulate unevenly during the cycle, prone to dendrite formation, and pose serious safety hazards.
A useful solution to problem 1 is to introduce 2% (mass fraction) LiF into the LLZTO system. The introduced LiF does not affect the crystal structure of LLZTO, but it can reduce the Li-Al-O grain boundary phase, water and CO2 of LLZTO. The response of LLZTO effectively reduces the interface resistance between LLZTO and metallic lithium and the grain boundary resistance of LLZTO.
There are two main ways to deal with Problem 2: One is to introduce a polymer or gel electrolyte as a buffer layer between the metal lithium and the electrolyte interface to infiltrate the metal lithium and suppress the lithium dendrites. The other is to sputter a substance that can form an alloy with metal lithium on the electrolyte ceramic sheet. The sputtered layer can spontaneously form an alloy layer with lithium during the cycle, and then reach a good touch between the lithium and the electrolyte surface, reduce the interface impedance, and suppress The composition of lithium dendrites.
2.1.2 Perovskite-type Li3xLa2/3-xTiO3 solid electrolyte
Perovskite-type (LLTO) electrolyte has the advantages of stable structure, simple preparation process, and wide range of variable composition. The room temperature particle conductivity of LLTO reaches 10-3S/cm, but its grain boundary resistance is relatively large. The ion conductivity of pure phase LLTO is less than 10-5S/cm, which reduces its total conductivity.
The main problem of LLTO solid electrolyte is its low total conductivity and poor stability with the metal lithium negative electrode.
The total conductivity of LLTO is mainly controlled by the conductivity of the grain boundary. The doping of Li/La sites and Ti sites can improve the conductivity of the particles, but has little effect on the conductivity of the grain boundaries. Grain boundary retouching improves the conductivity of the data more. it works.
When amorphous SiO2 is introduced into the LLT matrix, the total conductivity at 30°C reaches 1×10-4S/cm.
The stability between LLTO and metal lithium negative electrode is poor, because metal lithium can partially reduce Ti4+ to Ti3+ and introduce electronic conductance. Solution: Coat the surface of the LLTO with a solid polymer electrolyte to prevent the LLTO from touching the metal Li directly. The assembled all-solid-state battery has excellent cycling performance.
2.1.3 NASICON solid electrolyte
NASICON-type solid electrolyte has high ionic conductivity and excellent stability to water and air, and then attracted wide attention of researchers.
The NASICON solid electrolyte needs to deal with the problem of the touch surface of the motor and the occurrence of lithium dendrites.
WEN et al. reported a gel-ceramic multilayer electrolyte based on NASICON-type Li1.5Al0.5Ge1.5(PO4)3 (LAGP), which can effectively improve the interface touch.
The GOODENOUGH team reported a polymer/ceramic/polymer sandwich electrolyte. The polymer has good wettability with metal lithium, so the lithium ions at the interface can be uniformly accumulated, and then the occurrence of lithium dendrites can be suppressed. The ceramic piece Li1.3Al0.3Ti1.7(PO4)3 (LATP) in the middle allows only lithium ions to pass through and does not allow anions to pass through, that is, the migration number is 1, which reduces the potential difference at the interface between polymer and lithium metal. More stable.
2.1.4 Anti-perovskite solid electrolyte
The inverse perovskite structure solid electrolyte has the characteristics of low cost, environmental friendliness, high room temperature ionic conductivity (2.5×10-2S/cm), excellent electrochemical window and thermal stability, and stability with metal Li. Li3-2xMxHalO where M is a high-valent cation such as Mg2+, Ca2+, Sr2+ or Ba2+, and Hal is element Cl or I.
The anti-perovskite solid electrolyte currently under study is Li3ClO. In the anti-perovskite structure Li3ClO, Cl atom occupies the body center of the cube, O atom occupies the center of the octahedron, and Li+ ion occupies the apex of the octahedron; forming a significant Li-rich structure. After being doped with high-valent cations (such as Mg2+, Sr2+, Ca2+, Ba2+), the presence of cations makes a large number of vacancies in the crystal lattice. This increases the transmission channel of lithium ions, reduces the activation energy of Li+ ion dispersion, and improves the ionic conductivity of the electrolyte.
2.1.5 LiPON thin film solid electrolyte
LiPON materials have excellent comprehensive functions. The room temperature ion conductivity is 2.3×10-6S/cm, the electrochemical window is 5.5V (vs.Li/Li+), and the thermal stability is good. It is compatible with LiCoO2, LiMn2O4 and other positive electrodes and metals. Lithium, lithium alloy and other negative electrodes have good compatibility.
The seminar on LiPON materials focuses on its preparation methods.
CHINHO et al. used Li(C11H19O2), (C2H5)3PO4 and ammonia as materials to synthesize LiPON materials using the metal-organic chemical vapor deposition method (MOCVD). The room temperature ion conductivity can reach 2.95×10-7S/cm.
OUDENHOVEN et al. found that the LiPON film prepared by MOCVD has good compatibility with the Si anode, which can effectively suppress the formation of the anode SEI film and improve the cycle life.
NISUL et al. used the atomic layer deposition (ALD) method to prepare a LiPON film with a higher N element content (the composition is Li0.95PO3.00N0.60), and the room temperature ion conductivity was increased to 6.6×10-7S/cm.
The researchers also chose element substitution and partial substitution to prepare LiPON-type amorphous electrolytes with better functions.
JOO et al. chose S to replace P to prepare a compound with Li0.29S0.28O0.35N0.09 component, referred to as LiSON, with an ionic conductivity of 2×10-5S/cm and an electrochemical stability window of 5.5V.
2.2 Sulfide solid electrolyte
Compared with O, the binding effect of S on Li is weaker, which is conducive to the transfer of Li+. Therefore, the conductivity of sulfides is often significantly higher than that of oxides of the same type. Many main group elements can form stronger covalent bonds with sulfur, and the resulting sulfide is more stable and does not react with metal Li, making the sulfide electrolyte have better chemical and electrochemical stability. The sulfide electrolyte is sensitive to water vapor in the air and is unstable to metal lithium. It may still be pierced by lithium dendrites at high currents. The contact with the electrode deteriorates rapidly when the applied pressure is removed. [7]
Ohtomo et al. found that adding FeS, CuO and other additives to the 75Li2S-25P2S5 electrolyte system can significantly suppress the occurrence of H2S gas.
In 1999, Kanno et al. proposed to replace the oxygen in LISICON with sulfur to obtain a crystalline solid electrolyte with a thio-LISICON structure.
Kamaya et al. reported a sulfide crystalline electrolyte Li10GeP2S12 (LGPS) with three-dimensional lithium ion dispersion channels, and its room temperature conductivity reached 1.2×10-2S/cm.
Kato et al. developed a new type of sulfide crystalline electrolyte Li9.54Si1.74P1.44S11.7Cl0.3, which has an ion conductivity of 2.5×10-2S/cm at 27°C.
The sulfide glass solid electrolytes include Li2S-SiS2 and Li2S-P2S5, and their intrinsic conductivity is only 10-8~10-6S/cm. Hayashi et al. found that after high-temperature crystallization treatment, part of the Li2S-P2S5 glass phase crystallized to form glass ceramics, and the two-phase structure made the electrolyte conductivity significantly improved. In addition, the introduction of a new type of network structure into the sulfide glass electrolyte by doping can also improve the conductivity of lithium ions.
2.3 Polymer solid electrolyte
The composition of the polymer electrolyte (SPE) is similar to that of the organic electrolyte, except that the solvent of the polymer electrolyte exists in solid form. Compared with electrolyte batteries, polymer solid-state batteries have the following advantages: ①Good thermal stability, good safety function, and can work at 60-120℃ for a long time without burning and blasting; ②It can be made into flexible thin-film batteries.
The problem with polymer solid electrolytes is the low ionic conductivity at room temperature, and it is generally required to work at a temperature higher than its melting temperature, but the mechanical strength drops again after the temperature rises. An urgent problem in polymer electrolytes is the contradiction between high ionic conductivity and high mechanical strength. In order to deal with this contradiction, the researchers have done a lot of work.
ARCHER et al. reported a polyethylene/polyethylene oxide (PE/PEO) cross-linked polymer electrolyte. The PE chain provides mechanical strength, and the PEO chain conducts lithium ions. Then it reaches a balance between ionic conductivity and mechanical strength. The cross-linked SPE has both high ionic conductivity (1.6×10-4S/cm at 25°C) and high mechanical strength.
KANG et al. polymerized polyvinyl alcohol with cyanoethyl side chain and succinonitrile on polyacrylonitrile fiber membrane in situ to obtain a polymer with higher electrical conductivity (0.3S/cm) and mechanical strength (15.31MPa) Electrolyte (SEN).
GUO, etc. cleverly designed a class of dual-function polymer electrolytes with high mechanical strength and ionic conductivity through photopolymerization. The electrolyte is a polyether-acrylic acid interpenetrating network (ipn-PEA) combined with polyethers. The flexibility and rigidity of polyacrylic acid make ipn-PEA have both high mechanical strength (12GPa) and high room temperature ionic conductivity (0.22mS/cm).
Yang et al. designed a three-dimensional gel polymer electrolyte that is superior to liquid electrolytes. It has high ionic conductivity and mechanical strength, and can also suppress the ability of lithium dendrites. The resulting metal lithium battery has excellent electrochemical performance.
The researchers also tested the preparation of new polymer solid electrolytes: CUI et al. prepared a new polyvinylidene carbonate (PVCA) electrolyte. PVCA has a wide electrochemical stability window (4.5V) and suitable ionic conductivity (50%). 9.82×10-5S/cm at ℃).
2.4 Composite solid electrolyte
Compared with pure polymer solid electrolyte, composite solid electrolyte has a lower melting temperature (Tm) and glass transition temperature (Tg). The presence of the filler improves the ionic conductivity and mechanical function of the electrolyte, and the electrolyte is stable and compatible with the lithium negative electrode.
CUI et al. reported a composite electrolyte obtained by compounding Y2O3 doped ZrO2 nanowires with PAN. The nanowires are enriched with positively charged oxygen vacancies. These positive charges can interact with lithium ions to promote the dissociation of lithium salts and make the composite The electrolyte has a conductivity of 1.07×10-5S/cm.
CUI et al. mixed Li0.33La0.557TiO3 (LLTO) nanowires obtained by electrospinning with polyacrylonitrile (PAN) to obtain a composite electrolyte. LLTO nanowires form a useful ion-conducting network in the polymer matrix and significantly promote ion conductivity. The room temperature ion conductivity is 2.4×10-4S/cm.
The above-mentioned materials are obtained through physical mixing method of organic-inorganic composite electrolyte, and can also be obtained through organic-inorganic materials.
In the future, JUNLEE Energy, which has been committed to battery research and development, is a challenge and an opportunity. The R&D team of engineers will provide the world with more economical new energy batteries, and will improve lithium-ion battery technology to reduce the total cost.
JUNLEE Group is an integrated full power energy factory that specializes in Uninterruptible Power Supply (UPS), Lead-Acid Battery, Battery pack, EV battery, Energy Storage Battery, Energy storage power station, Power pack Gel battery, PV Inverter and Solar system.
Production capacity reach 200000 KVaH per month. Products apply to Electric vehicles,electric mobility, solar & wind energy storage system, UPS, backup power, telecommunication, medical equipment and lighting.
JUNLEE sets up "Power research center" with more High-tech products.More than 100 engineers provided in-time and efficient one-stop solutions.
They mission strives to bring green power to the world.
To learn more about Li-ion batteries, please refer to https://www.junleepower.com/